Nanofluidics
Introduction
Nanoscale fluidic channels represent a new regime for studies of ionic transport and DNA dynamics. In this regime, the channel height is smaller than the radius of gyration of a typical piece of lambda-DNA. Depending on electrolyte concentration, it can also be smaller than the Debye screening length, the characteristic length of the diffuse layer of counter ions that screen surface charges. In this interesting regime, we have carried out experiments on surface-charge-governed ion transport, streaming currents, surface charge inversion in the presence of multivalent counter ions, pressure-driven transport of DNA, and the efficiency of hydrostatic to electric energy conversion. In future work, we plan to keep working in these fields as well as to extend our work to ionic transistors and fluidic transport through carbon nanotubes.
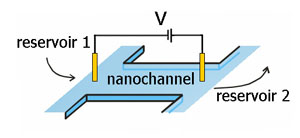
Slitlike nanochannels were used for our experiments. They have a typical height of 70 nm to 1 µm, a length of a few millimeters and a width of 50 to 200 microns. From two reservoirs, these channels can be filled with ionic solutions. By inserting electrodes in the reservoirs, a voltage can be applied over the nanochannel. Similarly, a pressure difference can be applied.
Device fabrication
The nanofluidic channels that we use in our experiments can be fabricated in two ways: Either we etch a groove in form of the channel into a chip and bond a second chip onto the first one to close the channel. Or we design the channel geometry out of a sacrificial material, enclose this completely using a different material and then etch the sacrificial layer away selectively to open the channel. The latter approach allows for the integration of electrodes.
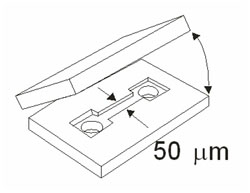
Channels can be fabricated by etching the channel geometry into a fused silica chip and bonding a second chip on top of the first one (left image). Another possibility (right image) is to first define the channel geometry using a sacrifical material (a), then enclosing it in a cover layer that contains holes (b) through which the sacrificial material is etched away (c). Closing the channel and creating two reservoirs (d) leads to the same channel geometry as the first method.
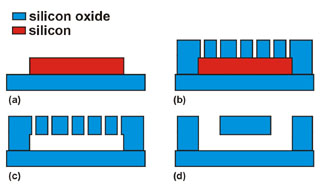
Nanofluidics – ion transport
Ion transport in nanofluidic channels
We found that ion transport in electrolyte solutions in nanochannels differs strongly from bulk behavior. In the dilute limit, electrical conductance saturates at a value that is determined by surface charge density, but independent of salt concentration and channel height.. The conductance in this limit can be influenced by surface modifications.
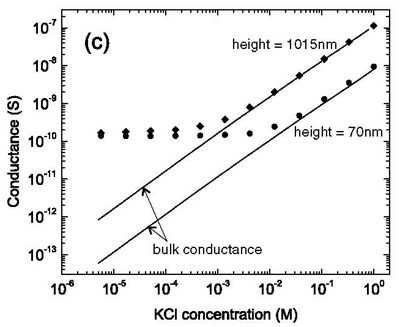
The conductance of two nanochannels with a height of 70 nm and 1015 nm, respectively, is depicted as a function of salt concentration. In the low-salt regime, the conductance deviates from bulk conductance (solid lines) and saturates at a value independent of channel height.
Streaming currents in nanochannels
A pressure-driven flow in a fluidic channel drags all ions with it. Owing to the screening ions in the Debye layer, a net charge transport is generated. This effect was studied in single nanochannels down to a height of 70 nm. The generated current is proportional to the pressure gradient and increases with channel height. It is constant below ~10 mM and decreases with higher salt concentrations. Using the Navier-Stokes equation and Poisson-Boltzmann theory, the streaming current can be related to the effective surface charge. This makes streaming currents in nanochannels suitable to investigate the effects of multivalent ions on the effective surface charge. Multivalent ions can “overscreen” the surface charge and thus cause a change in sign of the effective surface charge. This so-called charge inversion was observed for divalent (Ca2+, Mg2+) ions at concentrations higher than 400 mM and for trivalent (cobalt(III)sepulchrate) ions at concentrations higher than 100 µM. For the latter, the addition of monovalent ions (KCl) in a high concentration destroys the charge inversion.
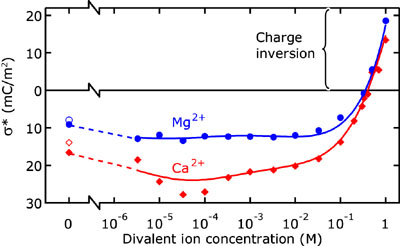
Effective surface charge as calculated from streaming current measurements as a function of salt concentration (Mg2+ and Ca2+). Charge inversion is seen in the high-salt regime. Solid lines are a guide to the eye.
Pressure-driven electrical power generation
Knowing that a pressure-driven flow can generate currents and voltages, we were interested in the efficiency of this hydrostatic to electrical power conversion. A theoretical investigation for a slit-like nanochannel of constant surface charge density predicts the maximum conversion efficiency at low salt concentrations. However, the experimentally observed conversion maximum is lower than theoretically predicted. This can be explained taking into account additional losses coursed by electrical conductance in the Stern layer.
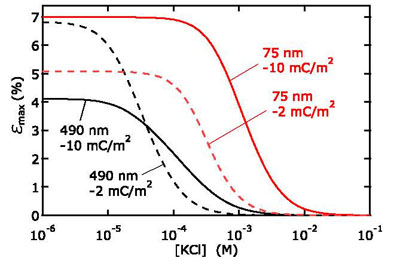
Theoretical calculations of the maximal attainable energy conversion efficiency as function of salt concentration for different channel heights and surface charges. For KCl ions, about 7 % was the calculated maximum conversion efficiency.
DNA dynamics in nanochannels
Pressure-driven transport of individual DNA molecules was investigated for channels of different heights. For channels higher than a few times the molecule’s radius of gyration, DNA mobility increased with molecular length. In smaller confinements, however, the mobility was practically independent of the length of the DNA (Figure 6). Additionally, we found that Taylor dispersion is greatly reduced for DNA molecules in small confinements. All this behavior can be understood regarding the DNA as a coil that is performing a random flight in the parabolic flow profile of the pressure-driven flow. Our further studies in this field aim for understanding the DNA conformational dynamics in two-dimensional confinements.
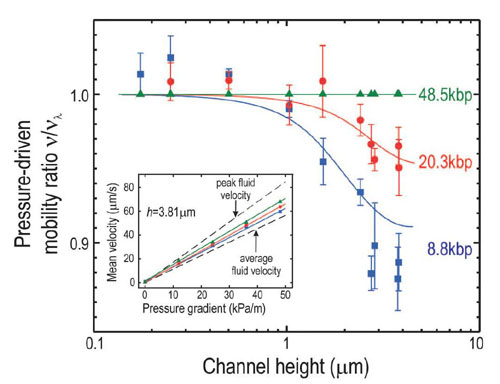
The pressure-driven mobility of DNA is shown as a function of the channel height for different lengths of the DNA molecules. Mobilities are scaled with respect to the mobility of lambda DNA, solid lines represent theoretical calculations. The pressure-driven mobility is defined as the slope of the molecule’s velocity as a function of pressure gradient (inset). For large channel heights, this mobility decreases with the size of the molecules as can be seen form the inset. For smaller channels, however, it reaches a common value independent of the length of the molecule.
The dynamics under pressure-driven flow of a lambda DNA molecule confined to a nanochannel of 90 nm height is shown.